Christine Chesley with Samer Naif and Kerry Key
LDEO, Columbia University
Because New Zealand’s north island lies at the juncture between the converging Pacific and Indo-Australian plates, it is not surprising that the area experiences earthquakes. A unique feature of the Hikurangi margin, the name of New Zealand’s subduction zone, is that its earthquake slip behavior varies from north to south along strike. The northern Hikurangi margin is characterized by shallow slow slip events (SSEs) and weak seismic coupling while the southern margin exhibits deeper SSEs and stronger coupling. The host of other properties that change along this subduction zone have motivated the question, “What controls the along-strike variation in megathrust behavior at the Hikurangi margin?”
One key element of this question lies in quantifying the porosity and fluid budget along the margin. Marine electromagnetic (EM) methods are well-suited for imaging fluids and fluid pathways within the lithosphere. Of course, a major caveat to any geophysical survey of convergent margins is the challenge of collecting good data on the seafloor beneath a deep ocean. So that is what we set out to do on 16 December 2018.
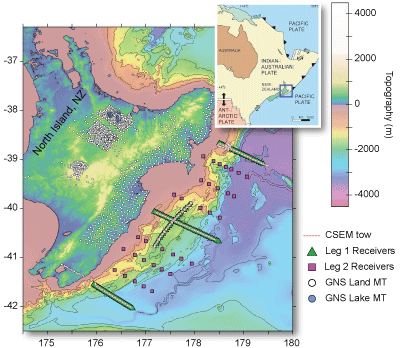
Figure 1. Survey map showing location of leg 1 OBEM deployments (green triangles), leg 2 OBEM deployments (magenta squares), CSEM tows (peach lines), and GNS land receivers (white and blue circles). Inset shows regional tectonics (from http://volcano.oregonstate.edu).
“We know about earthquakes here in Wellington,” asserted a waiter at the Thistle Inn. After a satisfying meal, my colleague and I were giving an abridged rundown of our cruise objectives to this excited employee. It was a day or so before we would leave for a month-long voyage to deploy ocean bottom electromagnetometers (OBEMs) for controlled-source electromagnetic (CSEM) and magnetotelluric (MT) imaging of the subseafloor off New Zealand’s north island. Curious about our business in New Zealand, our waiter warned us that talking about earthquakes was making people anxious in his country. Somehow, it was refreshing to find a non-geophysicist who thought our work was important. But it also impressed upon me the urgency to make this cruise a success.
The cruise itself was divided into two legs, both of which were carried out on the R/V Roger Revelle. The first and longer of the two legs involved the collection of the four lines of CSEM data shown in Figure 1, in addition to the deployment of 42 OBEMs for collection of passive MT data. I had never been to sea for more than a few hours – as a geophysics PhD student, I would spend most of my days in front of a computer rather than performing manual labor. I am pretty accustomed to having stable ground beneath my feet and a bed that doesn’t rock at night.
Everything about the experience was new for me.
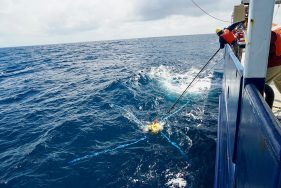
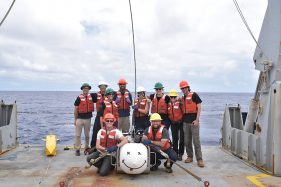
Before the cruise, I had only ever read about how our marine EM group collects data. Getting a firsthand look at the process has given me a tremendous amount of respect for how much effort goes into data collection, especially when things don’t go according to plan.
During the first leg of the cruise, the science crew consisted of eight researchers – five PhD students, two postdocs, and our Principal Investigator, Samer Naif, who led this cruise for the first time as Chief Scientist. The crew also included two Scripps EM Lab technicians and two Research Technicians to operate the cranes and supervise our actions on deck, making sure we were following safety protocols. Each twelve-hour shift counted six extremely hardworking individuals. Steady seas and mild to warm weather persisted for the majority of the first cruise, helping us ease into our sea legs and avoid seasickness.
Though we faced noteworthy obstacles in securing each line of CSEM data, the first line has given every one of us an answer to that age old interview question on describing a challenge we overcame. We began by deploying 38 of the Scripps OBEMs in just 24 hours, a nontrivial task as only five members of our entire team had ever assembled these receivers before the cruise. Receivers are the heart and soul of any data collection survey, and the Scripps OBEMs are broadband systems that continuously measure the horizontal components of natural and induced electromagnetic field energy. Such energy propagates through the Earth’s lithosphere in a manner that should depend on its electrical conductivity, which in turn depend in part on variations in fluid content. Proper assembly of the receivers is the first step to ensuring quality data recovery. I appreciated the inexhaustible patience shown by our Scripps EM Lab technicians, Jake Perez and Chris Armerding. From explaining to re-explaining how to use a torque wrench, test the acoustics on our receivers, properly affix electrodes, or attach a concrete block to the base of the receiver, Jake and Chris transformed our group of mostly inexperienced grad students into capable field workers. They showed us the multifaceted usefulness of 3M Scotch 35 electrical tape and cable ties that held electrodes, copper, or wires in place and always seemed to find a home in the pockets of my work pants.
Still jet-lagged and adjusting to twelve hours of manual labor per day, the first line of deployments was the most taxing. Nevertheless, the successful deployment of the receivers provided some reprieve as the next step was to tow our active source instrument, SUESI, the Scripps Undersea Electromagnetic Source Instrument. SUESI’s sharklike body tows behind it both long (~300 m) and short (~10 m) antennas terminated by thirty meter copper electrodes. By attaching SUESI to the ship’s winch using a standard oceanographic 0.680” coaxial deep-tow cable, we can send an alternating electric current from the ship to SUESI. SUESI then rectifies the signal and converts it from high voltage to a high current rectangular waveform that gets injected into the seawater across the copper electrodes. Thus, SUESI’s antennas behave as an EM dipole whose energy propagation can be used to probe the shallow lithosphere. As we started deploying SUESI, Poseidon decided it was time to pay for the nice weather and brisk pace we had enjoyed until then. After the arduous process of assembling, deploying, and lowering SUESI into the depths of the ocean, one of her copper antennas partially snapped. We had to haul SUESI back on board, repair the antenna, and deploy her down into the ocean again, a process that took several hours of deckwork. Hopefully, that was enough excitement to last the entire month. But no. The next day brought with it an inexplicable malfunction that led to yet another retrieval of SUESI. Perhaps she did not like the west Pacific water all that much. Thankfully, our Chief Scientist Samer Naif and lab techs Jake and Chris had planned for the unexpected and brought SUESI’s sister along, as a spare. We had better luck with the second SUESI and ended up relying on her for the remainder of the cruise.
Upon recovering SUESI at the end of the tow, it was time to retrieve the OBEMs to use them for the second line. Even with a heavy concrete block to carry the receivers to the seafloor (Fig. 1), ocean currents can move the OBEMs laterally away from the drop site during their descent through the water column. Once on the seafloor, it is necessary to know the exact location of the OBEM to accurately model the CSEM data. This is achieved by measuring the time it takes for an acoustic pulse sent from the ship to be repeated by the OBEM receiver. Similar to a game of “Marco Polo,” the ship sends and receives these acoustic signals at multiple locations until we have enough information to deduce where the receiver resides. We then send a specially coded acoustic signal to release the OBEM from its concrete block. Once the receiver floats to the surface, the team must act quickly to fish it out of the water. For me, retrieving the surfaced OBEMs was the most nerve wracking part of the process. What if we didn’t throw the grappling hook far enough? What if we couldn’t hook the receiver to the crane? What if the GPS buoy malfunctioned and the receiver couldn’t be located? Despite these worries, we managed to recover every single OBEM that we deployed for CSEM data, not only for the first line but for each of the next three as well – a total of 128 stations.
And what beautiful data we retrieved.
Between steak nights and fish tacos, rom coms and Coen Brothers movies, podcasts on olive oil and speculations about giant squids breaking our instruments, we collected three more lines of CSEM data following a similar routine of deploy-tow-recover. We learned to tie bowlines, clove hitches, and square knots. We watched sunrises, sunsets, witnessed dolphins playing with the bow and participated in safety drills of varying theatrics. And when all was said and done, we would manage to gather 20% more CSEM data than initially planned.
With the CSEM portion of the cruise over, we deployed all 42 OBEMs for the passive source MT portion of the project. Though broadband OBEMs can simultaneously collect CSEM and MT data, we left the receivers on the seafloor for about one month to collect higher quality, long-period MT data. This allowes us to look deeper into the Earth to learn about the lithosphere-asthenosphere system.
The second leg of the cruise in February 2019 involved recovering the OBEMs from the MT deployment phase. This leg included thirteen participants, five of whom were based in New Zealand. Though I did not participate in the second cruise, I was thrilled to hear that all 42 receivers were recovered despite the gnarly weather the team encountered. Taken together with the first cruise, it means a perfect recovery rate for all 170 deployments.
Combined data with the land MT sites collected by GNS Science, New Zealand, this is the largest amphibious EM dataset to date. I am thrilled to be working on this tremendous amount of data for the remainder of my PhD and excited to find what secrets they will unlock about the nature of the Hikurangi margin.
>> It was very educating and fun to work with instruments other than the ones I am used to from my institute. I also took home some ideas for organizing science on research vessels, which might benefit my work group.
– Gesa Franz
>> Even when the waves were high and we could surf in a chair inside the Roger Revelle it was an amazing personal and scientific experience. In my particular case, as a person used to coding and doing mathematics, to do ‘real’ science was very inspiring.
– Julen Alvarez-Aramberri
>> Doing fieldwork at sea gave me a whole new sense of what it means to do science, to be a scientist. It is so much more than analyzing or modeling data on a computer in the mundane safety of an office. We were out on deck in 40 knot winds and six meter seas. Science tests your body and your resolve, not just your mind. Just being on a research vessel dedicated solely to advancing our understanding of our amazing planet was inspiring. And then, of course, there were the sunrises, the stars, and the dolphins.
–Daniel Blatter
Reference information